Nuclear Physics Applications Through the Lens of Undergraduate Research
Nuclear physics is a broad and nuanced field that often is not well understood by the general public. Frequently in discussing my research with friends and family, I find that using the word nuclear can be enough to either prompt a separate discussion on nuclear weapons and meltdowns or, worse, make their eyes glaze over in pure boredom. Furthermore, there is a lack of accessible literature on the topic, making nuclear physics even more nebulous to the public. Most may not realize that discoveries in nuclear physics are the basis of life-saving technologies such as radiotherapy, cancer research, medical imaging, and smoke detectors. Nuclear technologies keep us safe by their use in export-control tracking of radioactive and dangerous materials across our borders. And discoveries in nuclear physics have propelled our understanding of fundamental questions like, "What fuels the sun?" and, "How did our universe come to be?"
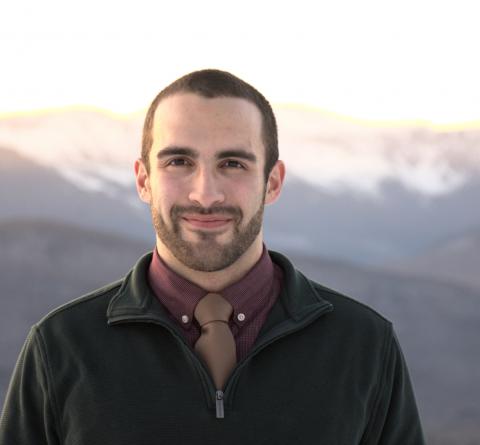
Tristan Anderson
I first became fascinated with nuclear physics back in 2013, not long after the first observation of the Higgs boson. The Higgs boson was predicted in the late 60s by Peter Higgs; his theory explained that elementary particles within the constituents of atoms needed to have mass. To me, the notion of questioning where mass came from seemed absurd, but like any kid in 2013, I turned to Google to start me on my way. The findings of that literature search exposed me to a completely new way of conceptualizing the universe and led me to study physics in college. In Dr. Karl Slifer’s laboratory at the University of New Hampshire (UNH), I conducted nuclear physics research under a Department of Energy grant and a 2018 Undergraduate Research Award (URA) from the Hamel Center for Undergraduate Research.
In this commentary, I will give an overview of nuclear physics. Then, I will share what motivated my research in a specific area called polarized target physics, and will also share what I have learned about practical applications of nuclear physics. I hope that by the end of this commentary readers will recognize how the field of nuclear physics contributes to our lives through technologies we rely on.
What Is Nuclear Physics?
Nuclear physics is an age-old study, and it arose from a curiosity about the natural world originating in Greek philosophy nearly 2,500 years ago. Around 500 B.C., Leucippus (a natural philosopher) guessed that the material world consisted of finite and indivisible particles, which we call atoms. The world accepted various forms of atomism until the late 1800s and early 1900s, when experimental evidence suggested that atoms were in fact divisible.
By 1911, Ernest Rutherford discovered the nucleus of the atom, and just a few years later, protons and neutrons (called nucleons) were discovered as components of the nucleus (Rutherford, 1911). By the mid-1920s physicists discovered spin: an intrinsic form of angular momentum in particles. By the late 1960s, Stanford physicists found that nucleons (particles within an atom’s nucleus) contained even smaller particles, called quarks. Bound systems of quarks are called hadrons, and understanding how the quarks within nucleons interact is an important step in understanding nucleons and nuclei as a whole (Povh et al., 2015). Today, small and large collaborations between theoretical and experimental physicists all over the world work to determine the fundamental properties and behavior of matter by inventing, sharing, or reusing complicated experimental techniques involving tools such as particle-accelerators and/or large detector arrays.
My Experience
Since my first year of college, I have been doing research with the UNH polarized target group. I have researched polarized target physics within Dr. Karl Slifer’s lab, and there, in collaboration with Jefferson and Fermi National Laboratories, the polarized target group contributes to answering one of the longest-standing problems in nuclear physics: How does spin arise in the proton?

The polarized target group at UNH. From left to right: the author, Dr. Elena Long, Michael McClellan, Dr. Nathaly Santiesteban, Dr. Karl Slifer, David Ruth.
Since the 1920s, physicists have been able to observe spin, which is just a fundamental form of angular momentum that exists within certain particles (Gerlach & Stern, 1922). Spin can be observed at many scales: in nuclei, as in the nucleus of carbon-13; in hadrons such as protons or deuterons; and in elementary particles such as quarks (Povh et al., 2015). Some physicists invented technologies that utilize spin, such as magnetic resonance imaging (Lauterbur, 1973) and nuclear magnetic resonance (Breit & Rabi, 1931; Purcell, 1945), but today, physicists are still searching for the origin of spin and how it influences matter on all scales in which it is observed. Results from nuclear physics scattering experiments are key to finding out how spin arises in particles such as the proton. Scattering experiments consist of three things: (1) a particle accelerator, (2) a target (my research at UNH involved creating a particular type of target), and (3) a detector.
In a scattering experiment, a particle accelerator shoots particles (usually electrons) in a beam at a stationary target. The target could be any piece of matter. Often, frozen-gas targets like solid ammonia are used; otherwise, frozen alcohol solutions, metals, and low-density gaseous targets can also be used. When the beam of particles hits the target, the result is projectile “scatter.” Depending on the experiment, the scatter consists of particles from the beam that bounce off the target as well as particles from the target itself. Finally, a detector measures the attributes of the scattered projectiles.
Detectors are complex instruments that measure the momentum of the scattered projectile (or the product of mass, speed, and direction); the location of the projectile when it is detected; and the energy of the projectile. Using these pieces of information across millions of detected particles, nuclear physicists create a “cross-section” that describes the distribution of scattered particles. They can analyze the cross-sections mathematically to learn about structures and properties of matter, such as the presence of quarks or the spin of particles. Results from scattering experiments have and will continue to improve physics theories, reveal properties of matter that technologies could harness, and improve models that can answer questions about the origins of the universe or some of the most exotic states of matter, such as neutron-stars.

Our work in Dr. Slifer’s lab at UNH creates polarized target materials for scattering experiments conducted with some of the equipment pictured here, at the US Department of Energy’s Jefferson Lab Hall A in Newport News, Virginia. Photo courtesy of Jefferson Lab.
The polarized target group at UNH is involved in scattering experiments. Polarized targets are targets in which a high degree of nuclear spins are aligned within an external magnetic field. Our group creates and polarizes targets for scattering experiments that take place at national research laboratories, such as the Department of Energy’s Jefferson Lab in Virginia. A collaboration of more than one hundred people, including the UNH group, collect data from polarized target scattering experiments, and then physicists analyze the collected data and conclude various nuances to the nuclear medium. Nuclear spin is at the core of the research done in the polarized target group. We use nuclear magnetic resonance spectroscopy as a tool to measure the degree of nuclear polarization (the degree to which nuclear spin is aligned) in a material.
Readers are probably familiar with one of the practical applications of nuclear magnetic resonance in the medical field. Three-dimensional nuclear magnetic resonance signals can be compressed into flat images, which is known as magnetic resonance imaging, or MRI. An MRI is a non-invasive imaging technique that traditionally uses the spins of the hydrogen in the water of your body to produce an image. MRI images are like a heat map for the concentration of spins in a region of your body. Traditional MRI technology that uses the spins of hydrogen in a person’s body is excellent at resolving large internal structures within the body, but it is not effective at tracking biological processes in real time. However, over the past twenty years, researchers and medical professionals have started using pre-polarized materials, instead of the hydrogen in a person’s body, as contrasts for MRI procedures. An MRI contrast acts like a dye; much like hydrogen, it is a source of nuclear spins that help form an MRI image. Pre-polarization of MRI contrasts greatly improves their effectiveness (Wolber et al., 2004). Pre-polarized contrasts enhance the nuclear magnetic resonance signal by two to three orders of magnitude, which lends itself to higher resolution images (Wolber et al., 2004). With this increase in signal strength and careful selection of MRI contrast, it is possible to image metabolic processes in living organisms, not only in a higher definition but also in real time.
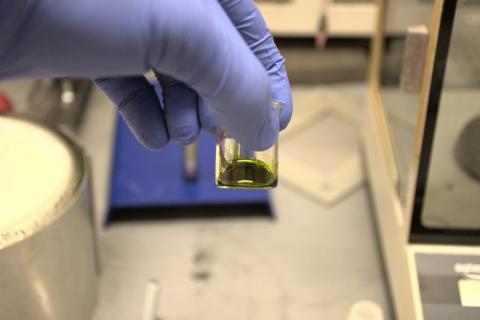
The C13 target material the author made, shown here in a 10 mL beaker, could potentially be used as a contrast to be ingested by someone undergoing an MRI procedure.
Dynamic nuclear polarization is an effective technique used to align the spins of particles in the direction of an external magnetic field. The polarized target group works with this polarization technique at UNH, and it dramatically boosts the nuclear magnetic resonance signals that I analyzed for the sake of our nuclear physics research. Fortunately, the boost in nuclear magnetic resonance signal strength is useful in MRIs. Furthermore, the polarization technique also has utility in the pre-polarizing of MRI contrasts used by medical professionals for MRIs. This technique allows the metabolization of molecules containing carbon-13 that are detected in detailed MRI images of cellular metabolic processes. That research is external to UNH. But, both as a means of becoming more connected to the research behind developmental medical imaging technology and as an in-vitro study to test the UNH polarized target group's material polarization capabilities, I made a carbon-13-based target identical to what is sometimes used as an MRI contrast by medical professionals, by following the techniques used in Wolber et al. (2004). This application of the dynamic nuclear polarization technique is extremely useful in the study of cancer, as it broadens the avenues in which diagnostic medical imaging can occur. Within the last twenty years, physicists and medical professionals have carefully studied this additional contrast preparation. Today, technology exists to prepare and pre-polarize MRI contrasts automatically.
Other Applications of Nuclear Physics
Nuclear physics is an enormous field of study, laden with many subfields beyond what I have studied so far, that all have far-reaching applications in our daily lives. The effect of nuclear physics on today’s society is subtle, but it is everywhere.
Precision atomic clocks are useful for a variety of reasons, such as GPS, data coincidence for time-sensitive observations in radio astronomy, and gravitational-wave detection. The technology in modern smoke detectors evolved from the study of nuclear decay (Povh, 2014). In some smoke detectors, radiation is released and used to detect the attenuating presence of smoke. Radiation is also used to sterilize laboratory equipment and disposable hospital equipment. For example, nose-swabs, single-use syringes, and surgical gloves are all sterilized with some form of radiation during and after their manufacturing process. But don’t worry, the type and amount of radiation used means that these applications are not harmful.

The Radura insignia is placed on foods that have been radiated.
Another sterilization application of radiation is in our foods. But you won’t be radiated by those either; all foods that are radiated in this process are inspected for radiological contamination, which is quite easy to detect. If you are curious to know which foods you consume are exposed to this treatment, keep an eye out for the Radura insignia that appears on labels of food products.
Homeland security and international nuclear non-proliferation organizations take interest in gamma-ray spectroscopy, the study of the energy spectrum of gamma rays that are released from radioactive materials, for regulating the export of radioactive materials. Through a special type of particle detector called a scintillator, customs and export control can identify radioactive materials in extremely small quantities. All that a federal agency needs to do to detect radiation of this type is pass each package, suitcase, and person through an array of these passive detectors at every seaport, airport, or border (Doyle, 2011).
Conclusion
During my studies at the University of New Hampshire, I became a passionate researcher in nuclear physics and find myself fascinated with its applications in medicine and emergent nuclear technologies. What stood out to me the most from my experience was that a regular undergraduate could become a contributor in a good-sized collaboration between several research labs at the University of New Hampshire and a handful of universities around the world. My undergraduate years have been the most formative and perspective-broadening period of my life. In fall of 2021, I will be enrolled in a nuclear physics Ph.D. program at Virginia Tech so that I can pursue a lifelong dream of impactful, life-saving research.
Work done under DOE grant DE-FG02-88ER40410, and a 2018 Undergraduate Research Award from the Hamel Center for Undergraduate Research. Special thanks to Dr. Karl Slifer and Dr. Ellie Long for your eternal patience, words of wisdom, and support over the past five years. Thank you for showing me how to effectively do research, how to program, and how to analyze data. I am grateful for your support and humbled to have been a part of the UNH polarized target group. Family, friends, and colleagues, I hope you understand the depth of my gratitude for keeping me motivated in my pursuit of higher education and research. I am indebted to you, and I will never forget that. This article is dedicated to J.A.K: Never give up.
Bibliography
Breit, G. and Rabi, I.I. Measurement of Nuclear Spin. Phys. Rev. 38, 2082–2083, (1931). https://doi.org/10.1103/PhysRev.38.2082.2.
Doyle, J. Nuclear Safeguards, Security, and Nonproliferation. Burlington, MA: Elsevier, (2011).
Gerlach, W. and Stern, Otto. Der experimentelle Nachweis der Richtungsquantelung im Magnetfeld. Z. Phys. 9, 349–355 (1922).
Lauterbur, P. Image Formation by Induced Local Interactions: Examples Employing Nuclear Magnetic Resonance. Nature 242, 190–191 (1973). https://doi.org/10.1038/242190a0.
Overhauser, Albert W., Polarization of Nuclei in Metals. Phys. Rev. 92, 411–415 (1953). https://doi.org/10.1103/PhysRev.92.411.
Povh, B. et. al. Particles and Nuclei: An Introduction to the Physical Concepts, 7ed. Berlin: Springer, (2014). https://doi.org/10.1007/978-3-662-46321-5.
Purcell, E.M. et. al. Resonance Absorption by Nuclear Magnetic Moments in a Solid. Phys. Rev. 69, 37–38 (1945).
Rutherford, E. The Scattering of Alpha and Beta Particles by Matter and the Structure of the Atom. Philosophical Magazine 21, 669–688 (1911).
Wolber, J. et. al. Generating highly polarized nuclear spins in solution using dynamic nuclear polarization. NIMA 526:1–2, 173–181 (2004). https://doi.org/10.1016/j.nima.2004.03.171.
Author and Mentor Bios
Tristan Anderson graduated from the University of New Hampshire (UNH) in December 2020 with a bachelor of science degree in physics and minors in applied mathematics and in French. He conducted research throughout his undergraduate years at UNH, culminating in his senior thesis. Tristan’s interest in physics was sparked back when he was in ninth grade and heard about the discovery of the Higgs boson. He explains that as he began studying physics, he realized how little he knew, and how much there was to understand about how the universe works. In Dr. Slifer’s lab at UNH, Tristan found “the constant baton-passing between theory and experiment” to be “humbling and exciting.” By writing his commentary, he hopes to promote what nuclear physics has to offer and “kindle a spark of interest” in Inquiry readers and prospective physicists alike. Tristan will soon head to Virginia Tech in Blacksburg, Virginia, to study toward his Ph.D., and after that, he aims to work in research and development in nuclear physics. Eventually he would like to return to academia to teach at the college level and share his belief in the importance of research with future students.
Karl J. Slifer began working at the University of New Hampshire (UNH) in 2008 and is an associate professor in the physics and astronomy department. His area of specialty is studying the internal structure of the proton and how its properties, particularly spin, arise from quarks and gluons and their interactions. Dr. Slifer notes that his research developing gas and then solid spin-polarized targets for scattering experiments has been conducted over many years, and he says he has been “fortunate to be able to work with an excellent team to help push this effort forward.” Over those years he has mentored about a dozen undergraduate researchers. Tristan is both the first of these mentees to write for Inquiry and his most productive research mentee. It is particularly exciting, Dr. Slifer explains, that Tristan has worked on synthesizing polarizable materials that could have crossover applications in the medical field. He is glad that Tristan has taken the opportunity to write for Inquiry and describes its value with a quote from Albert Einstein, "If you can’t explain it simply, you don’t understand it well enough.”
Copyright 2021, Tristan Anderson